The coronavirus in a tiny drop
By Carl Zimmer, New York Times
last updated: Dec 10,2021
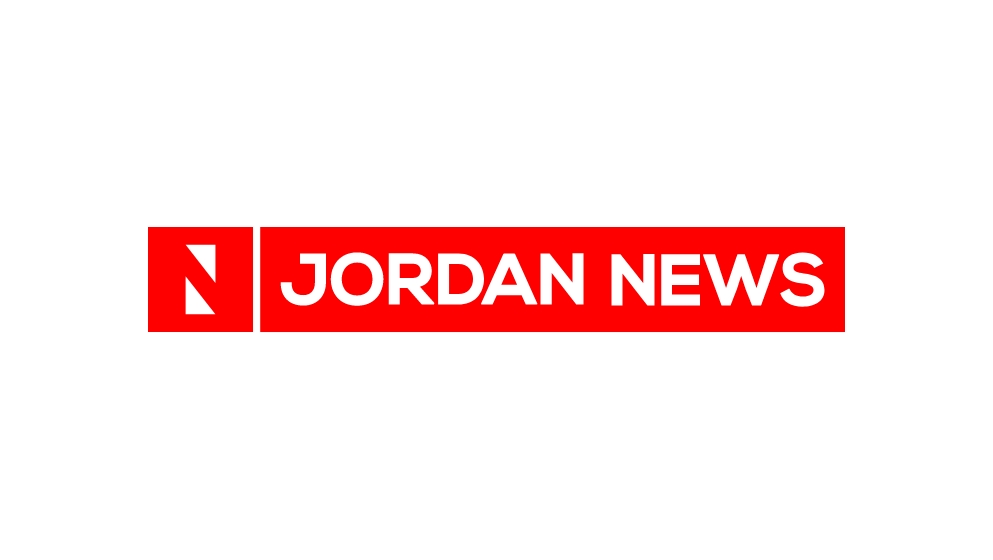
To better understand the coronavirus’s journey from one person
to another, a team of 50 scientists has for the first time created an atomic
simulation of the coronavirus nestled in a tiny airborne drop of water.
To create the model, the researchers needed one of the world’s
biggest supercomputers to assemble 1.3 billion atoms and track all their
movements down to less than a millionth of a second. This computational tour de
force is offering an unprecedented glimpse at how the virus survives in the
open air as it spreads to a new host.
“Putting a virus in a drop of water has never been done before,”
said Rommie Amaro, a biologist at the University of California San Diego who
led the effort, which was unveiled at the International Conference for High
Performance Computing, Networking, Storage and Analysis last month. “People
have literally never seen what this looks like.”
How the coronavirus spreads through the air became the subject
of fierce debate early in the pandemic. Many scientists championed the
traditional view that most of the virus’s transmission was made possible by
larger drops, often produced in coughs and sneezes, which usually travel only a
few feet before dropping to the floor.
But epidemiological studies showed that people with COVID-19
could infect others at a much greater distance: Just talking in a poorly
ventilated space like a bar, church or classroom was enough to spread the
virus.
Those findings pointed to much smaller drops, called aerosols,
as important vehicles of infection. Scientists define droplets as having a
diameter greater than 100 micrometers, or about four-thousandths of an inch.
Aerosols are smaller — in some cases so small that only a single virus can fit
inside them. And thanks to their minuscule size, aerosols can drift in the air
for hours.
Viruses cannot survive forever in aerosols, though. Researchers
often find that viruses collected from the air have become so damaged that they
can’t infect cells anymore. It’s possible that as the aerosols evaporate, the
air destroys the virus’s molecular structure. Or the chemistry inside the tiny
drop may become too hostile for them to survive.
“At this point, we don’t understand how that happens,” said
Linsey Marr, a professor of civil and environmental engineering at Virginia
Tech who was not involved in the new study. Microscopes that can capture
detailed images of what goes on inside a virus-laden aerosol have yet to be
invented.
In March 2020, Amaro and her colleagues decided the best way to
open this black box was to build a virus-laden aerosol of their own.
The researchers started by creating a model of the coronavirus,
known as SARS-CoV-2, from 300 million virtual atoms. They combined thousands of
fatty acid molecules into a membrane shell, then lodged hundreds of proteins
inside. Some of these proteins are important because they keep the membrane
intact. Others, called spike proteins, form flowerlike structures that rise far
above the surface of the virus. The tips of the spikes sometimes spontaneously
flick open, allowing the virus to latch on to a host cell and invade.
After building their virus, Amaro and her colleagues made an
aerosol to put it in. Using 1 billion atoms, they created a virtual aerosol
measuring a quarter of a micrometer in diameter, less than a hundredth the
width of a strand of human hair.
The researchers could not simulate the aerosol as a blob of pure
water, however. When an aerosol breaks free from the fluid in our lungs, it
brings along a stew of other molecules from our bodies.
That stew includes mucins: long, sugar-studded proteins from a
lung’s lining. Aerosols also carry molecules called surfactants, which keep the
delicate branches of our airways from sticking together. Charged atoms, like
calcium and magnesium, fly around the aerosol, exerting powerful forces on
molecules they encounter.
Once the virus was loaded into an aerosol, the scientists faced
the biggest challenge of the project: bringing the drop to life. Amaro and her
colleagues calculated the forces at work across the entire aerosol, taking into
account the collisions between atoms as well as the electric field created by
their charges. They determined where each atom would be four-millionths of a
billionth of a second later.
To carry out this vast set of calculations, the researchers had
to take over the Summit Supercomputer at the Oak Ridge National Laboratory in
Tennessee, the second-most-powerful supercomputer in the world. Because the
machine was in high demand, they could run their simulation only a few times.
“We only have so many shots to actually see if we can get this thing to
actually fly,” Amaro said.
The first run was a disaster. Tiny flaws in their model caused
the virtual atoms to crash into one another, and the aerosol instantly blew
apart. “It basically explodes,” Amaro said.
After half a dozen rounds of adjustments, the aerosol became
stable. The researchers ran the calculations all over again to see what
happened inside the aerosol an instant later. All told, they created millions
of frames of a movie capturing the aerosol’s activity for ten-billionths of a second.
“While molecular modeling is not a new thing, the scale of this
is next level,” said Brian O’Flynn, a postdoctoral research fellow at St. Jude
Children’s Research Hospital who was not involved in the study.
The buzzing activity Amaro and her colleagues witnessed offered
clues about how viruses survive inside aerosols. The mucins, for example, did
not just wander idly around the aerosol. The negatively charged mucins were
attracted to the positively charged spike proteins.
Amaro speculated that the mucins act as a shield. If the virus
moves too close to the surface of the aerosol, the mucins push them back in, so
that they aren’t exposed to the deadly air. “What we think is that it’s
actually covering itself in these mucins, and that’s acting like a protective
coating for it during flight,” Amaro said.
This discovery may help explain how the delta variant became so
widespread. Delta’s spike proteins have a more positive charge than those on
earlier forms of the coronavirus. As a result, mucins huddle more closely
around them. That attraction could potentially make the mucins a better shield.
Amaro and her colleagues are making plans to build an omicron
variant next and observe how it behaves in an aerosol. They want to wait for
structural biologists to work out the three-dimensional shape of its spike
proteins before getting started. But just looking at the early findings about
omicron, Amaro already sees one important feature: “It is even more positively
charged,” she said.
Because omicron’s spike proteins are even more positively
charged than delta’s, it may build a better mucin shield in aerosols. And that
may help make it even more transmissible.
Read More Lifestyle
(window.globalAmlAds = window.globalAmlAds || []).push('admixer_async_509089081')
(window.globalAmlAds = window.globalAmlAds || []).push('admixer_async_552628228')
Read More
Iron Levels May Explain Brain Fog During Menopause
5 Negative Effects of Eating Too Much Protein
The Top Drink to Eliminate Belly Fat: Discover It
To better understand the coronavirus’s journey from one person
to another, a team of 50 scientists has for the first time created an atomic
simulation of the coronavirus nestled in a tiny airborne drop of water.
To create the model, the researchers needed one of the world’s biggest supercomputers to assemble 1.3 billion atoms and track all their movements down to less than a millionth of a second. This computational tour de force is offering an unprecedented glimpse at how the virus survives in the open air as it spreads to a new host.
“Putting a virus in a drop of water has never been done before,” said Rommie Amaro, a biologist at the University of California San Diego who led the effort, which was unveiled at the International Conference for High Performance Computing, Networking, Storage and Analysis last month. “People have literally never seen what this looks like.”
How the coronavirus spreads through the air became the subject of fierce debate early in the pandemic. Many scientists championed the traditional view that most of the virus’s transmission was made possible by larger drops, often produced in coughs and sneezes, which usually travel only a few feet before dropping to the floor.
But epidemiological studies showed that people with COVID-19 could infect others at a much greater distance: Just talking in a poorly ventilated space like a bar, church or classroom was enough to spread the virus.
Those findings pointed to much smaller drops, called aerosols, as important vehicles of infection. Scientists define droplets as having a diameter greater than 100 micrometers, or about four-thousandths of an inch. Aerosols are smaller — in some cases so small that only a single virus can fit inside them. And thanks to their minuscule size, aerosols can drift in the air for hours.
Viruses cannot survive forever in aerosols, though. Researchers often find that viruses collected from the air have become so damaged that they can’t infect cells anymore. It’s possible that as the aerosols evaporate, the air destroys the virus’s molecular structure. Or the chemistry inside the tiny drop may become too hostile for them to survive.
“At this point, we don’t understand how that happens,” said Linsey Marr, a professor of civil and environmental engineering at Virginia Tech who was not involved in the new study. Microscopes that can capture detailed images of what goes on inside a virus-laden aerosol have yet to be invented.
In March 2020, Amaro and her colleagues decided the best way to open this black box was to build a virus-laden aerosol of their own.
The researchers started by creating a model of the coronavirus, known as SARS-CoV-2, from 300 million virtual atoms. They combined thousands of fatty acid molecules into a membrane shell, then lodged hundreds of proteins inside. Some of these proteins are important because they keep the membrane intact. Others, called spike proteins, form flowerlike structures that rise far above the surface of the virus. The tips of the spikes sometimes spontaneously flick open, allowing the virus to latch on to a host cell and invade.
After building their virus, Amaro and her colleagues made an aerosol to put it in. Using 1 billion atoms, they created a virtual aerosol measuring a quarter of a micrometer in diameter, less than a hundredth the width of a strand of human hair.
The researchers could not simulate the aerosol as a blob of pure water, however. When an aerosol breaks free from the fluid in our lungs, it brings along a stew of other molecules from our bodies.
That stew includes mucins: long, sugar-studded proteins from a lung’s lining. Aerosols also carry molecules called surfactants, which keep the delicate branches of our airways from sticking together. Charged atoms, like calcium and magnesium, fly around the aerosol, exerting powerful forces on molecules they encounter.
Once the virus was loaded into an aerosol, the scientists faced the biggest challenge of the project: bringing the drop to life. Amaro and her colleagues calculated the forces at work across the entire aerosol, taking into account the collisions between atoms as well as the electric field created by their charges. They determined where each atom would be four-millionths of a billionth of a second later.
To carry out this vast set of calculations, the researchers had to take over the Summit Supercomputer at the Oak Ridge National Laboratory in Tennessee, the second-most-powerful supercomputer in the world. Because the machine was in high demand, they could run their simulation only a few times. “We only have so many shots to actually see if we can get this thing to actually fly,” Amaro said.
The first run was a disaster. Tiny flaws in their model caused the virtual atoms to crash into one another, and the aerosol instantly blew apart. “It basically explodes,” Amaro said.
After half a dozen rounds of adjustments, the aerosol became stable. The researchers ran the calculations all over again to see what happened inside the aerosol an instant later. All told, they created millions of frames of a movie capturing the aerosol’s activity for ten-billionths of a second.
“While molecular modeling is not a new thing, the scale of this is next level,” said Brian O’Flynn, a postdoctoral research fellow at St. Jude Children’s Research Hospital who was not involved in the study.
The buzzing activity Amaro and her colleagues witnessed offered clues about how viruses survive inside aerosols. The mucins, for example, did not just wander idly around the aerosol. The negatively charged mucins were attracted to the positively charged spike proteins.
Amaro speculated that the mucins act as a shield. If the virus moves too close to the surface of the aerosol, the mucins push them back in, so that they aren’t exposed to the deadly air. “What we think is that it’s actually covering itself in these mucins, and that’s acting like a protective coating for it during flight,” Amaro said.
This discovery may help explain how the delta variant became so widespread. Delta’s spike proteins have a more positive charge than those on earlier forms of the coronavirus. As a result, mucins huddle more closely around them. That attraction could potentially make the mucins a better shield.
Amaro and her colleagues are making plans to build an omicron variant next and observe how it behaves in an aerosol. They want to wait for structural biologists to work out the three-dimensional shape of its spike proteins before getting started. But just looking at the early findings about omicron, Amaro already sees one important feature: “It is even more positively charged,” she said.
Because omicron’s spike proteins are even more positively charged than delta’s, it may build a better mucin shield in aerosols. And that may help make it even more transmissible.
Read More Lifestyle
To create the model, the researchers needed one of the world’s biggest supercomputers to assemble 1.3 billion atoms and track all their movements down to less than a millionth of a second. This computational tour de force is offering an unprecedented glimpse at how the virus survives in the open air as it spreads to a new host.
“Putting a virus in a drop of water has never been done before,” said Rommie Amaro, a biologist at the University of California San Diego who led the effort, which was unveiled at the International Conference for High Performance Computing, Networking, Storage and Analysis last month. “People have literally never seen what this looks like.”
How the coronavirus spreads through the air became the subject of fierce debate early in the pandemic. Many scientists championed the traditional view that most of the virus’s transmission was made possible by larger drops, often produced in coughs and sneezes, which usually travel only a few feet before dropping to the floor.
But epidemiological studies showed that people with COVID-19 could infect others at a much greater distance: Just talking in a poorly ventilated space like a bar, church or classroom was enough to spread the virus.
Those findings pointed to much smaller drops, called aerosols, as important vehicles of infection. Scientists define droplets as having a diameter greater than 100 micrometers, or about four-thousandths of an inch. Aerosols are smaller — in some cases so small that only a single virus can fit inside them. And thanks to their minuscule size, aerosols can drift in the air for hours.
Viruses cannot survive forever in aerosols, though. Researchers often find that viruses collected from the air have become so damaged that they can’t infect cells anymore. It’s possible that as the aerosols evaporate, the air destroys the virus’s molecular structure. Or the chemistry inside the tiny drop may become too hostile for them to survive.
“At this point, we don’t understand how that happens,” said Linsey Marr, a professor of civil and environmental engineering at Virginia Tech who was not involved in the new study. Microscopes that can capture detailed images of what goes on inside a virus-laden aerosol have yet to be invented.
In March 2020, Amaro and her colleagues decided the best way to open this black box was to build a virus-laden aerosol of their own.
The researchers started by creating a model of the coronavirus, known as SARS-CoV-2, from 300 million virtual atoms. They combined thousands of fatty acid molecules into a membrane shell, then lodged hundreds of proteins inside. Some of these proteins are important because they keep the membrane intact. Others, called spike proteins, form flowerlike structures that rise far above the surface of the virus. The tips of the spikes sometimes spontaneously flick open, allowing the virus to latch on to a host cell and invade.
After building their virus, Amaro and her colleagues made an aerosol to put it in. Using 1 billion atoms, they created a virtual aerosol measuring a quarter of a micrometer in diameter, less than a hundredth the width of a strand of human hair.
The researchers could not simulate the aerosol as a blob of pure water, however. When an aerosol breaks free from the fluid in our lungs, it brings along a stew of other molecules from our bodies.
That stew includes mucins: long, sugar-studded proteins from a lung’s lining. Aerosols also carry molecules called surfactants, which keep the delicate branches of our airways from sticking together. Charged atoms, like calcium and magnesium, fly around the aerosol, exerting powerful forces on molecules they encounter.
Once the virus was loaded into an aerosol, the scientists faced the biggest challenge of the project: bringing the drop to life. Amaro and her colleagues calculated the forces at work across the entire aerosol, taking into account the collisions between atoms as well as the electric field created by their charges. They determined where each atom would be four-millionths of a billionth of a second later.
To carry out this vast set of calculations, the researchers had to take over the Summit Supercomputer at the Oak Ridge National Laboratory in Tennessee, the second-most-powerful supercomputer in the world. Because the machine was in high demand, they could run their simulation only a few times. “We only have so many shots to actually see if we can get this thing to actually fly,” Amaro said.
The first run was a disaster. Tiny flaws in their model caused the virtual atoms to crash into one another, and the aerosol instantly blew apart. “It basically explodes,” Amaro said.
After half a dozen rounds of adjustments, the aerosol became stable. The researchers ran the calculations all over again to see what happened inside the aerosol an instant later. All told, they created millions of frames of a movie capturing the aerosol’s activity for ten-billionths of a second.
“While molecular modeling is not a new thing, the scale of this is next level,” said Brian O’Flynn, a postdoctoral research fellow at St. Jude Children’s Research Hospital who was not involved in the study.
The buzzing activity Amaro and her colleagues witnessed offered clues about how viruses survive inside aerosols. The mucins, for example, did not just wander idly around the aerosol. The negatively charged mucins were attracted to the positively charged spike proteins.
Amaro speculated that the mucins act as a shield. If the virus moves too close to the surface of the aerosol, the mucins push them back in, so that they aren’t exposed to the deadly air. “What we think is that it’s actually covering itself in these mucins, and that’s acting like a protective coating for it during flight,” Amaro said.
This discovery may help explain how the delta variant became so widespread. Delta’s spike proteins have a more positive charge than those on earlier forms of the coronavirus. As a result, mucins huddle more closely around them. That attraction could potentially make the mucins a better shield.
Amaro and her colleagues are making plans to build an omicron variant next and observe how it behaves in an aerosol. They want to wait for structural biologists to work out the three-dimensional shape of its spike proteins before getting started. But just looking at the early findings about omicron, Amaro already sees one important feature: “It is even more positively charged,” she said.
Because omicron’s spike proteins are even more positively charged than delta’s, it may build a better mucin shield in aerosols. And that may help make it even more transmissible.
Read More Lifestyle